The physiology of physical exercise is known as exercise physiology. This allied health profession focuses on the acute reactions and long-term adaptations to physical activity. The most skilled practitioners in the field of exercise, exercise physiologists use education, lifestyle modification, and targeted exercise programs to treat and recover from both acute and long-term illnesses and injuries.
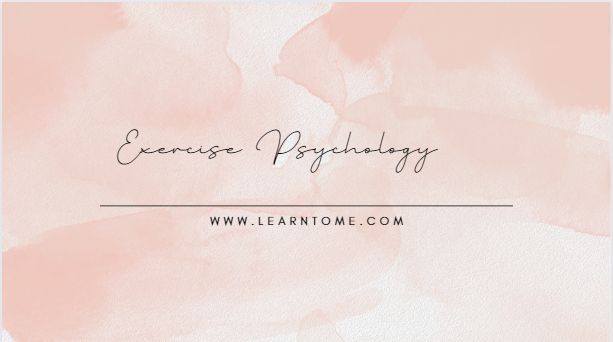
Studying particular alterations in the neurohumoral, cardiovascular, and muscular systems that result from strength or endurance training in changes in functional ability and strength is essential to comprehending the impact of exercise.[2] The body’s response to exercise-induced adaptive reactions has been described as the training effect or as “an elevation of metabolism produced by exercise” [3].[4]
History of Exercise physiology
In 1922, the British scientist Archibald Hill established the ideas of oxygen debt and maximal oxygen uptake.[5][6] For their separate contributions to the field of muscular energy metabolism, Hill and German physician Otto Meyerhof were awarded a share of the 1922 Nobel Prize in Physiology or Medicine.[7]
Building on these findings, researchers started calculating the amount of oxygen used during exercise. Among others who made significant contributions were the Harvard Fatigue Laboratory, German universities, the Copenhagen Muscle Research Centre, Scandinavian academics Per-Olof Åstrand and Bengt Saltin in the 1950s and 1960s, and Henry Taylor at the University of Minnesota.[8][9]
It serves as a primary health care provider in several nations. University-trained specialists known as Accredited Exercise Physiologists (AEPs) prescribe exercise-based therapies with customized dosage response prescriptions to treat a range of diseases.
Energy expenditure
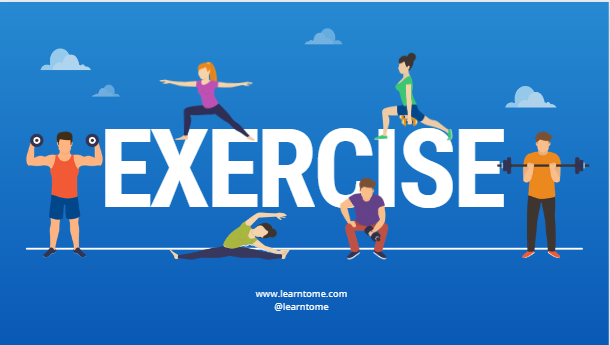
People are highly capable of exerting themselves continuously for extended periods of time. For instance, a single rider cycling 8,204 km (5,098 mi) at 26.4 km/h (16.4 mph) for 50 consecutive days used 1,145 MJ (273,850 kcal; 273,850 dieter calories) with an average power output of 173.8 W.[10]
When engaged in continuous action (e.g., repeatedly extending the human knee), skeletal muscle consumes 90 mg (0.5 mmol) of glucose per minute. This results in approximately 24 W of mechanical energy and, because muscle energy conversion is only 22–26% efficient, approximately 76 W of heat energy. The basal metabolic rate, or resting energy consumption, of skeletal muscle is 0.63 W/kg[13], which means that the energy consumption of passive and active muscles differs by a factor of 160.
Energy expenditure for brief muscle contractions can be significantly higher: an adult male person can mechanically produce 314 W/kg when springing up from a squat. This kind of quick movement can produce twice as much in nonhuman creatures like bonobos and certain small lizards [14].[15]
When compared to the adult human body’s basal resting metabolic rate, this energy expenditure is significantly higher. This rate is generally between 45 W to 85 W, while it fluctuates slightly depending on size, gender, and age.[16] [17] The amount of energy expended by muscles throughout a given day determines the total energy expenditure (TEE), which is significantly higher.[18] Hence, exercise controls the body’s energy metabolism, especially if it is done consistently for extended periods of time.
Metabolic changes
Rapid energy sources
Unlike aerobic respiration, which uses oxygen and is sustainable and takes place in the mitochondria, anaerobic metabolism happens in the cytosol of muscle cells and provides the energy required for brief, high-intensity bursts of activity. Adenylate kinase, rapid glycolysis, and the phosphocreatine (PCr) system make up the quick energy sources. The universal energy source found in all cells, adenosine triphosphate (ATP), is synthesized again by each of these processes.
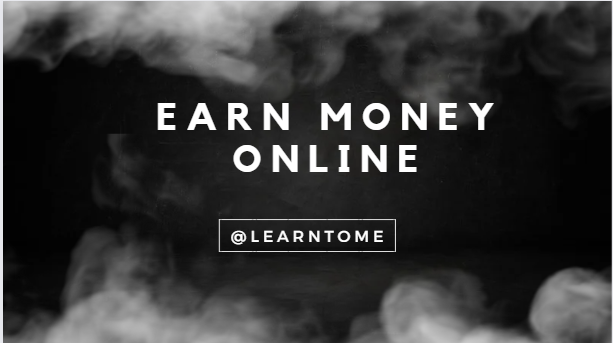
The PCr system, which uses the enzyme creatine kinase, is the fastest source of the aforementioned sources, but it is also the one that is most easily exhausted. Phosphocreatine and adenosine diphosphate (ADP) are combined by this enzyme to produce ATP and creatine.
.. Because oxygen is needed for mitochondrial creatine kinase to resynthesise phosphocreatine, this resource has a limited shelf life. This substrate is therefore limited to a maximum of 10 to 30 seconds of high-intensity labor under anaerobic circumstances. Fast glycolysis, on the other hand, primarily employs intracellular glycogen as a substrate and can operate for around two minutes before becoming fatigued.
During vigorous activity, glycogen phosphorylase quickly breaks down glycogen into individual glucose units. After that, glucose is converted to lactic acid in anaerobic environments and oxidized to pyruvate. Acidosis is aided by this process, which converts NADH to NAD and releases a hydrogen ion. Fast glycolysis cannot be maintained over extended periods of time because of this.
Plasma glucose
When the rate of glucose appearance (input into the blood) and disposal (removal from the blood) is equal, plasma glucose is said to be maintained. In a healthy person, during moderately intense exercise, the rates of appearance and disposal are roughly equal.
However, prolonged or intense exercise can cause an imbalance that leans toward a higher rate of disposal than appearance, at which point glucose levels drop and fatigue sets in. The amount of glucose absorbed in the stomach and the amount of glucose produced by the liver (hepatic) determine the rate at which glucose appears.
While the liver can catabolize stored glucose, glucose appearance during exercise usually does not come from glucose absorption from the gut.
Because skeletal muscle, the second primary glycogen store, is unable to transfer glucose into the circulation through glycogenolysis, the liver’s ability to do so is unique. Glycogen phosphatase, an enzyme found in liver cells as opposed to skeletal muscle, takes a phosphate group off of glucose-6-P to liberate free glucose. This phosphate group must be removed for glucose to get through a cell membrane.
Gluconeogenesis contributes significantly to the liver’s glucose output, but it is insufficient to maintain exercise on its own. Because of this, during activity, when glycogen stores are depleted, glucose levels drop and weariness sets in. The other half of the equation, glucose disposal, is regulated by glucose uptake at the contracting skeletal muscles.
glucose control: As previously noted, insulin secretion is decreased during exercise and does not significantly contribute to the maintenance of a normal blood glucose concentration; however, the concentrations of its counter-regulatory hormones increase during exercise.
The three main ones are growth hormone, adrenaline, and glucagon. Among other things, all of these hormones increase the production of glucose by the liver (hepatic). For example, growth hormone and adrenaline both activate adipocyte lipase, increasing the release of non-esterified fatty acids (NEFAs). This preserves glucose consumption and aids in blood sugar maintenance during exercise by oxidizing fatty acids.
Exercise and diabetes: For people with diabetes mellitus, exercise is an especially effective means of controlling blood sugar levels. Moderate exercise can cause more glucose to be disposed of than to appear when blood glucose levels are elevated (hyperglycemia), which lowers total plasma glucose concentrations. As previously mentioned, this glucose disposal mechanism operates independently of insulin, making it very beneficial for individuals with diabetes.
Furthermore, it seems that an increase in insulin sensitivity occurs 12 to 24 hours after exercise. For people with type II diabetes who are producing enough insulin but have peripheral resistance to insulin signaling, this is especially helpful.
Those with diabetes should refrain from exercising during severe hyperglycemic episodes, though, as there may be consequences from ketoacidosis. Because exercise increases the synthesis of ketone bodies in response to elevated levels of circulating NEFAs, it may worsen ketoacidosis.
Obesity and type II diabetes are closely related, and type II diabetes may have something to do with the way fat is deposited in the liver, muscles, and pancreas. Most people who lose weight through diet and exercise also tend to have higher insulin sensitivity, probably because of this relationship.[20] This impact can be especially strong in certain individuals and lead to normal glucose regulation. People with diabetes can lead regular lives without having to worry about complications from the disease, even if there is no known cure; nonetheless, weight gain would undoubtedly ensue.
Oxygen
Exercise and hard labor are examples of vigorous physical activity that raises the body’s oxygen requirement. An rise in heart rate, breathing rate, and breathing depth is the body’s first response to this demand.
The Fick Equation, which says that the amount of oxygen consumed during exercise is equal to cardiac output (Q) multiplied by the difference between arterial and venous oxygen concentrations, provides the best description of oxygen consumption (VO2) during exercise: VO2=Q x (a-vO2diff). In layman’s terms, oxygen consumption is determined by the amount of blood the heart pumps out and the working muscle’s capacity to absorb the oxygen in that blood, however this is a little bit of an oversimplification.
.. VO2 max is determined by a number of factors other than cardiac output, which is regarded to be the limiting element in this relationship in healthy persons. That is, other aspects need to be taken into account, such the lung’s capacity to oxygenate blood. Conditions including diffusion limitation, ventilation/perfusion mismatch, and pulmonary shunts are caused by a variety of diseases and abnormalities that can restrict blood oxygenation and, consequently, oxygen distribution.
Additionally, a significant factor in the equation is the blood’s ability to carry oxygen. Exercise (ergogenic aids) that are used in endurance sports to raise the volume percentage of red blood cells (hematocrit), such as blood doping or the use of erythropoietin (EPO), frequently target the oxygen carrying capacity.
Dehydration
Both hypohydration (dehydration brought on by exercise) and exercise-induced dehydration (dehydration brought on by exercise) are terms used to describe dehydration. In addition to raising body temperature, heart rate, perceived exertion, and possibly increasing dependence on carbohydrates as a fuel source, the latter decreases aerobic endurance performance.
Athletes persisted in believing that fluid consumption was not useful for years after it was evident in the 1940s that exercise-induced dehydration had a negative impact on exercise performance. More recently, it has been shown that even mild (<2%) dehydration has detrimental effects on performance, and that exercising in a hot environment exacerbates these effects.
Depending on whether hypohydration is caused by diuretics or sauna use, which significantly lower plasma volume, or by previous exercise, which has a much smaller effect, the results can differ. Though its effects on muscle strength and endurance are inconsistent and call for more research, hypohydration lowers aerobic endurance.[21]
Sweat-based thermoregulation eliminates the metabolic waste heat produced by intense, continuous activity. In cool weather, a male marathon runner loses approximately 0.83 L per hour, but in warm weather, they lose 1.2 L (losses in females are about 68 to 73% lower).[22] Sweat loss during intense activity can be 2.5 times greater than pee loss.[23] Significant physiological repercussions may result from this.